Introduction
Hearing is one of the most important human senses, allowing the interpretation of air vibrations (sounds), being an essential part of the process of communication. This complex transduction of mechanical energy into bioelectrical energy is made by the ear, which is divided into the outer, middle and inner ear [1]. The outer ear consists of the ear pinna and the external auditory canal. This system drives vibrations toward the tympanic membrane. The middle ear transmits vibrations from the tympanic membrane to the oval membrane through a set of three ossicles, the malleus, incus, and stapes, this last one in direct contact with the oval membrane. The middle ear complex helps to overcome the low vibration transmission efficiency between two media, air to perilymph, in the inner ear. Finally, the inner ear is formed by the semicircular canals, vestibule and the cochlea, the latter being the structure responsible for the transformation of vibrations into nerve impulses that can be interpreted by the brain [2].
Inside the cochlea, the following structures can be distinguished: the scala vestibuli and the scala tympani, both containing perilymph (1–2 mM [K+]); and the scala media, which contains endolymph (150 mM [K+] and a endocochlear potential of 100–120 mV) and the organ of Corti [3, 4]. The scala media is separated from the scala vestibuli and the scala tympani by the Reissner’s and basilar membranes, respectively. Moreover, the stria vascularis and the spiral ligament can be found on the side wall of the scala media.
Vibrations are transmitted from the stapes to the perilymph in the scala vestibuli, through the oval membrane. Then, each vibrational frequency advance through the perilymph to reach their specific resonance area in the basilar membrane, where the maximum vibration level and focal stimulation are produced [5]. The vibration of the basilar membrane induce the opening of the mechanosensitive K+ channels, activating the inner hair cells (IHCs), initiating the impulse. Simultaneously, the outer hair cells (OHCs) are in contact with the tectorial membrane. They modulate and amplify the stimulus, allowing small vibrations to be amplified enough to stimulate IHCs [2]. High-frequency sounds stimulate areas closer to the oval membrane, while low-frequency sounds activate the apical areas. Primarily, IHCs transmit the stimulus. In fact these cells are innervated by spiral ganglion neurons (SGNs), mainly afferents, whereas OHC are innervated by fewer SGNs, mainly efferent.
Cell types and synapse systems
The main elements involved in the hearing processing are the hair cells (HCs), SGNs, supporting cells (SCs) and ribbon synapses. HCs have an elongated structure, with ciliated projections or stereocilia of varying sizes at the apical pole. The movement of one stereocilia is transmitted through cadherin-23/protocadherin-15 bonds connected to the K+ channel present in the next stereocilia. Thus, the movement induces channel opening and initiates the signal transduction (Figure 1 A) [6, 7]. The entry of K+ increases the membrane potential of HCs and allows the opening of Ca2+ channels, located in the basal side of the cell, which are involved in the release of glutamate vesicles (Figures 1 A, B).
Figure 1
Hair cell structure and function. Vibrations induce the opening of K+ channels located in the apical ciliated structures (stereocilia), leading to the K+ internalization (A). In response to the membrane potential depolarization, the basal Ca2+ channels open leading to the glutamate vesicles release to the synaptic cleft (B)
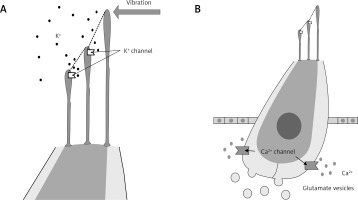
SGNs are excited by the glutamate molecules released by the HCs, initiating the nerve impulses that are ending in the brain. These neurons are the first element of the primary and non-primary auditory pathway, transmitted to different brain areas, where the signals are interpreted. The functional relationship between IHCs and type I SGNs is mainly mediated by the ribbon synapses, a system that enables fast and stable glutamate release from the presynaptic region, i.e., HCs. OHCs also establish ribbon synapses, but with type II SGNs; however, the density of these synapses is much lower and their function remains unknown [8, 9].
SCs are in direct contact with HCs providing physical and molecular support owing to the secretion of some factors [10]. SCs are also responsible for the recycling of K+, which is mediated by intercellular connexin junctions, allowing the K+ flow to reach the stria vascularis. This structure is responsible for the maintenance of the endolymph homeostasis, which is essential for the ear function and transduction of the vibrational stimuli [4, 11].
Hearing loss
More than 5% of the world population lives with some degree of hearing impairment (360 million people including 32 million children) according to the latest estimates of the World Health Organization (WHO, February 2013).
The main factors behind hearing degeneration are ototoxic drugs, aging, continued exposure to excessive noise and infections. After an injury, the auditory system is damaged irreversibly, because the regeneration system is inhibited or deactivated in higher mammals, oppositely to other non-mammalian vertebrates [12]. The pool of adult stem cells in the inner ear drops dramatically after birth. Therefore, an endogenous cellular source for regeneration is absent. In mammals, HCs are only generated during a short embryonic period; hence, their loss in adults produces an irreversible hearing defect. Similarly, the SGN degeneration is unrecoverable and in the case of synaptic loss, recovery has been shown to be limited [13].
Hearing impairments can be divided into sensorineural (SNHL) and conductive. Conductive hearing loss is mainly treated by surgery, showing good outcomes, while SNHL patients are managed with external devices of sound amplification (cochlear implants, auditory brainstem implants, etc.) and/or with pharmacological therapies with little success until the moment (corticosteroids, antibiotics, etc.). Because SNHL can emerge after the degeneration of different cochlear components, there are multiple targets that should be reached in order to resolve this kind of hearing impairment. HCs, SGNs, ribbon synapsis system, stria vascularis and spiral ligament are currently the most studied elements, with a particular focus on the HC and SGN research. Nowadays there is no functional therapy for SNHL. Current strategies are directed to the hearing partial recovery using cochlear implants in severe cases or glucocorticoids in moderate or mild SNHL cases [14, 15].
Regeneration research
Because of the drastic reduction in the number of stem cells in the inner ear after the neonatal period, the autonomous regenerating capacity is almost depleted. Therefore, many research groups have focused their efforts on developing stem cell-based treatments to restore HC, SGN and SC populations. There are many studies outside the hearing research field, where stem cells showed remarkable healing capacities, for example, tendon-to-bone regeneration [16].
Stem cells are undifferentiated cells with self-renewal capacity, in presence of the appropriate stimulus [17–19]. Pluripotent stem cells have the ability to differentiate into any cell type derived from the three embryonic lineages (endoderm, ectoderm and mesoderm), while adult stem cells can only differentiate into the major specialized cell types of its tissue or organ. The auditory regeneration field is mainly focus on embryonic stem cells (ES) [20], adult stem cells [21, 22], or induced pluripotent stem cells (iPSCs) [23, 24]. However, nowadays the main issues to be solved are the obtaining of a proper efficiency in the production of auditory stem cells and to demonstrate the utility and safety of these cells in a clinical context [25].
Experimentation in animal models with regenerative capacity, such as zebrafish or avian models, has shown that their auditory regeneration is guided by the same genetic pathways activated during embryonic development. That mechanism leads to HC or stereocilia regeneration by different mechanisms (Figure 2), that have aroused great interest for the development of novel therapies that can reconstruct these pathways in humans [12].
Figure 2
Hair cell (HC) and stereocilia regeneration. HC degeneration can affect the entire cell or can be restricted to the stereocilia. In some animal models with auditory regeneration capacity, supporting cells (SCs) can replace damaged HCs through mitosis and differentiation or by transdifferentiation and growth factor secretion. Figure modified from [15]
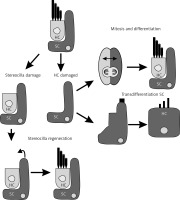
In this review, we focus on the conservation and regeneration of HCs by stem cells and complementary molecular therapies; delivery and integration of neural precursors and growth factors improving neuronal elongation and synaptic connections, for SGN regeneration; improving SCs survival and their transdifferentiation to HCs by molecular therapy; recent advances in delivery techniques to transplant stem cells into the inner ear; and monitoring techniques for auditory cell differentiation.
Advances in methodology for hearing regeneration
In our opinion, the important discoveries in this area are mainly focused on the development of methods for stem cell transplantation, improving migration, survival and new genetic systems for cell fate monitoring.
The supply of exogenous neural stem cells into the cochlea and their proper migration to the Rosenthal’s canal (RC) is a very complex process. Different routes for stem cell transplantation have been tested, such as through the perilymph [26, 27] or the endolymph [27]. Although these techniques are promising, their results show a low cell survival rate , with only small populations of new cells in the RC. Transplantation of cells into the modiolus (bone lamina inside the cochlea) or in the cochlear nerve, showed a higher cell survival rate and increased migration to the RC. However, the transplantation process involves potential hearing damage [28].
The direct transplantation of stem cells on the side wall tissue of the cochlea seems to achieve efficient results. The abundance of tissue and blood supply to the area, may be responsible for the increased survival of grafted cells in the wall, also improving migration of cells to the RC in both control mice and animals treated with ototoxic drugs. Moreover, it has been hypothesized that the basilar membrane has crevasses that would facilitate stem cell migration. This method induces the same temporary threshold shift of the auditory brainstem response (ABR), as the one observed in the classical transplantation through the scala tympani. Therefore, this method is as safe as the conventional methods of transplantation, but allowing a better cell survival and migration to the affected area [29].
Research on hair cells
One of the main research areas for hearing regeneration is focus on the production of new HCs, due to their importance in the auditory signal transduction. It has been postulated, that the precise knowledge concerning the genetic route responsible for the differentiation of stem cells to HCs, studied in vitro or in animal models showing hearing regeneration (Figure 3), could offer relevant clues to reveal the way to induce transdifferentiation of human SCs [7]. Most studies in this area have been conducted on avian models, which, unlike mammals, can regenerate the auditory epithelium [30]. The main disadvantage of this approach is that in the adult human ear, the stem cell population is virtually absent. Therefore, the only cells capable of supplying HCs are SCs. However, if SCs are transdifferentiated to HCs, the reduction in the SC population may influence HC survival, because of the depletion of secreted factors and disorganization of the organ of Corti’s structure. Consequently, transdifferentiation must be preceded by cell proliferation or by an external supply of stem cells [25].
Figure 3
Pathway for the stem cell differentiation into auditory neurons and hair cells. The scheme displays the step-by-step differentiation of embryonic or induced pluripotent stem cell, derived from placode precursors, to form cochlear neurons and hair cells. Cell intermediaries can be characterized by the marker expression profile specified in the figure. Figure modified from [45]
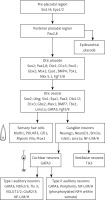
In this research field, ES [27, 25] and iPSCs [23. 24] have been widely used. ES have the advantage of maintaining a high proliferative capacity. However, they are difficult to obtain, generate an ethical debate, have tumorigenic potential and can elicit an immunologic response in the implanted subject [31]. For their part, iPSCs can be easily obtained from the somatic cells of the patient, and thus, no host rejection can be derived from cell autograft transplantation. Nevertheless, iPSCs have some disadvantages such as tumorigenicity, a reduced proliferation rate and the tendency to differentiate into the original somatic tissue [31]. The great potential of iPSCs and ES to produce derived HCs has been reported, but only in in vitro assays. Satisfactory results in graft implantation, or effective integration of differentiated HCs in the inner ear tissue in vivo have been rarely described [32–34]. This may be due to the complex cytoarchitecture and microenvironment of the organ of Corti, which is very different from the culture conditions in which the new HCs are generated.
Despite the above described problems, major improvements have been observed in the differentiation of ES and iPSCs to HCs, recording very promising results with the staggered method or step by step approach [32–34]. The study conducted by Chen et al. is noteworthy, as they describe a novel and effective stepwise differentiation method that allows the efficient otic precursors obtainment. The culture of stem cells in a specific medium containing fibroblast growth factor, led to the formation of epithelial progenitors, that can derive into SCs, HCs or neural progenitors [35]. Moreover, it has been suggested that culture conditions also influence the process, as for example, suspension cultures showed an improvement in the differentiation efficiency compared to adherent cultures [36]. Nevertheless, in vitro stem cell-derived HCs seem to exhibit the same mechanosensitive and electrical properties as immature inner ear HCs [37]. If so, these cells could induce a functional recovery in vivo, as long as they become adequately integrated into the target tissue.
Other interesting studies, in the HC topic, are focused on developing strategies to promote the protection or survival of damaged HCs, for example exposed to noise or ototoxic factors. Mesenchymal stem cells can be differentiated into fibrocytes and then transferred to the non-sensitive layer of the auditory epithelium, where they provide physical and molecular support for the damaged HCs [38]. Adipose-derived stem cells express certain neurotrophic factors, that can induce the regeneration or protection of HCs [39, 40]. In other cases, the aim of the intervention is to modulate the molecular microenvironment, for example, using IGF1, which reduces apoptosis and has protective effects [15, 41].
Research on spiral ganglion neurons
The hearing regeneration field had accomplish greater success in the SGN area, working with ES, iPSCs, neural stem cells (NSC) and neural precursor cells. As stated before, the only treatment nowadays for severe SNHL is the cochlear implant. This device is designed to circumvent the HCs or organ of Corti functional impairment, directly stimulating the SGNs through a set of electrodes [42, 43]. However, various factors can induce a SNHL with SGN degeneration, rendering the cochlear implant completely useless. These factors include some diseases (mumps, meningitis, multiple sclerosis, Meniere’s disease, etc.) or ototoxic damages. Additionally, some factors, as noise overexposure and aging, have been shown to be highly related with this pathology. In fact, both are responsible of the SGN death, or more usually the degeneration of the synapses established between the SGNs and HCs, which subsequently leads to a progressive death of the neurons that have lost their interaction [8, 44]. For these reasons, there has been a growing interest in the development of cellular therapies, increasing the number of functional SGNs that can be stimulated by cochlear implant electrodes [45].
Bone marrow stromal stem cells have shown an efficient cell migration and differentiation ability and nowadays they are considered a promising source of neuronal lineage cells [46]. Numerous experiments involving adult stem cells employed NSCs. In particular, the olfactory epithelium has been suggested as a good source of NSCs (oe-NSCs), which can generate new SGNs thanks to their good regenerative capacity in adult models [47, 48]. Moreover, oe-NSCs share some cell markers with some cell populations in the auditory epithelium. The implantation of oe-NSCs showed an improvement in the ABR, although the hearing damage was not sufficiently reduced [26, 29]. Interestingly, a recent study provided evidence of a new source of NSCs for this purpose: a purified subpopulation of glial cells expressing Sox2 that can be isolated from the auditory nerve [44].
Nowadays, many researchers defend the idea that the microenvironment generated after the degeneration of cochlear SGNs propitiates the homing, survival and differentiation of NSCs. In this context, stromal cell-derived factor-1 (SDF-1) may play an important role. Some studies suggest that SDF-1 is responsible for the migration of NSCs to the affected areas, based in the fact that after the injury, an overexpression of this factor (by the Schwann cells) and the augmentation of its receptor expression in the NSC are observed [49].
Step-by-step differentiation of ES and iPSCs, into otic or neuronal precursors, has been proposed (Figure 3) [45]. Thus, Chen et al. demonstrated a procedure for the production of neurons with a suitable phenotype, that lowers the ABR threshold, after their transplantation into deaf adult guinea pigs (Cavia porcellus) [35]. Several authors have confirmed the usefulness of NSCs derived from iPSCs [23] or ES [50, 28] for cell therapies. However, although novel neurons maintain a good survival ratio, both in vitro and in vivo, a large proportion of neurons seems incapable of emitting elongations or projections [51].
Finally, other strategies, without stem cells, have been developed in order to improve neural tissue restoration, by using different factors. Glial cell line-derived neurotrophic factor (GDNF) showed to induce increased branching capacities of the SGN neurites [52, 53]. In addition, GDNF promotes SGN survival, by specifically binding to the GDNF family receptor alpha 1 (GFRα1), activating PI3K/Akt and MEK/Erk phosphorylation pathways [53]. Cerivastatin is another active factor, involved in the elongation of the neurites [54], probably due to its inhibition of the post-translational modification of the Rho family proteins; this inhibition alters the normal function of the Rho family proteins, i.e., regulating the actin cytoskeleton and thus modulating the neurite elongation [55, 56]. However, despite the identification of some factors that promote the elongation and branching of neurons, in a large percentage of cases, no functional connections between SGNs and HCs have been observed until today.
Research on ribbon synapses
Noise and aging are the two principal factors implicated in the degeneration of the ribbon synapses connecting SGNs and HCs. Noise can induce permanent (PTS) or temporary threshold shift (TTS), depending on whether HCs are permanently damaged or not, respectively. However, some evidences suggest that successive TTSs lead to a PTS, mainly due to the synapse degeneration [8]. Although basal glutamate release is necessary to maintain synaptic connections [8, 57], the degeneration process may be a consequence of the excitotoxicity produced by excessive glutamate release in the presynaptic space, released by the HCs in response to continued intense noise exposure [8, 58]. Despite the fact, that the ABR threshold can be recovered after TTS, synapses and neural response amplitude cannot be recuperated (Figure 4).
Figure 4
Synaptic loss and ribbon synapse degradation. The ribbon synapse complex is organized so, those neurons with a low stimulation rate are concentrated in the modiolar site, while those with a higher stimulation rate are concentrated in the pillar site. Modiolar neurons are the most susceptible cells to noise- and aging-induced damage. Their synaptic degeneration leads to neuronal death. The remaining neurons modify their synaptic connection to compensate the neuronal population reduction. However, the process can be reversed, before the SGN death, with NT-3 and BDNF overexpression. Figure modified from [8]
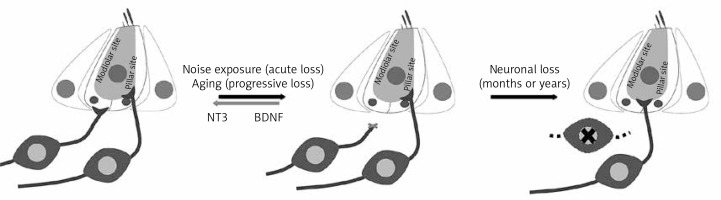
Also during aging, neuronal terminals display the same degeneration characteristics as those observed in noise traumas, suggesting that the ribbon complex degeneration can be also related with a glutamate over-release [59, 60]. However, the molecular mechanism is still unknown. It has been proposed that recurrent TTSs produce repeated glutamate excitotoxicity, leading to cumulative defects in the synaptic connections [8].
Although several experiments suggest that SGNs preserve spontaneous ability in vitro and in vivo to re-innervate the cochlea, the amount of new synaptic connections formed after denervation is lower than the normal pattern observed in non-denervated explants. Moreover, only a few implanted NSCs elongate and establish effective synapses [51]. This phenomenon may justify why a full hearing recovery has not yet been achieved.
Some of the most important issues in regenerative medicine for auditory regeneration, are the establishment of new functional ribbon synapses between novel neurons and/or HCs, and to avoid or reverse the conversion from TTS to PTS. However, mainly all the experimental strategies, developed until this day, are focused in stem cells or growth factors alone. In our opinion, the integration of stem cell implants, together with the growth factors already identified would enable great advances in this field.
During the embryonic stage, neurotrophin-3 (NT-3) and the brain-derived neurotrophic factor (BDNF) induce pro-survival and pro-elongation signals for SGNs [61]. NT-3 is expressed in all cells of the inner ear, in the postnatal state, and in the IHCs and SCs in the adult stage [62]. BDNF expression is limited to IHCs, OHCs and SCs in the postnatal state, whereas in adults, it is only expressed in SCs [63]. It has been shown that experimental therapies combining NT-3 and BDNF are able to increase the number of functional synapses, defined as the overlapping of the presynaptic (CtBP2 in HCs) and postsynaptic (PSD95 in SGNs) markers [51]. Another study clarified that BDNF is effective postnatally only in vestibular organs, where it is not expressed in limiting concentrations. Thus, BDNF may not be implicated in the maintenance of the organ of Corti. However, NT-3 acts as a limiting factor that influences the cochlear synaptic connections. NT-3 overexpression reduces the ABR thresholds, increases the amplitude of the ABR wave I (P1) and stimulates the regeneration of the ribbon synapses [10]. All this evidences suggests that auditory and vestibular neurons respond equally to NT-3 and BDNF during the embryonic stage, but the BDNF importance in the cochlea is lost after birth, being NT-3 the most relevant neurotrophic factor in the cochlea.
In addition to the explained neurotrophic factors, other interesting elements, named axonal guidance molecules, were proposed by Brugeaud et al. It has been hypothesized, that the ability to form new synapses between the SGNs and HCs is maintained even in adult stages. However, it is inhibited or blocked by axonal guidance molecules [64]. As an example, the repulsive guidance molecule a (RGMa) acts as an inhibitor of the neuronal fibers elongation [65]. This factor is expressed in the cochlear tissues and in the neuronal ganglion, in embryonic and adult stages, whereas its receptor, neogenin, is expressed only in the cochlear ganglion neurons. The RGMa knockdown by specific antibodies, increases the amount of new neuronal fibers and synapses in the organ of Corti explants. Furthermore, RGMa blockage showed to improve the pruning process, which is related to the maturation of cochlear neuronal connections (Figure 5) [64]. Therefore, the expression of RGMa in adults may be one of the main factors limiting synaptic regeneration between SGNs and HCs.
Figure 5
Synaptic pattern between hair cells (HCs) and spiral ganglion neurons (SGNs). Without in vitro blockage of repulsive guidance molecule A (RGMa; Control), only an erratic pattern of innervation is established after 18 days of HCs and SGNs co-culture (18 DIV). However, after in vitro blockage of RGMa, the cells develop an innervation pattern similar to in vivo neurons after 7 days (7 DIV). Figure modified from [64]
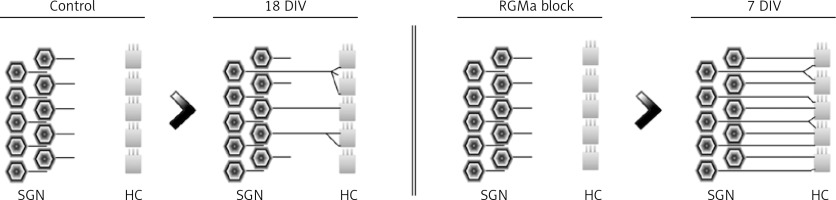
Future directions and clinical trials
The discovery of the spontaneous regeneration of HCs in birds [66] and the similarities between avian and human embryonic auditory development [67], promoted the development of a research field aiming to overcome the human auditory regeneration blockage.
In this review, we focused on reporting the current state of the regenerative research in hearing recovery. Stem cell research has enabled remarkable advances in regeneration, particularly in neuronal cells and synapses. Despite the progress achieved, there are certain issues that require a deeper investigation, in order to improve the results already obtained, or to develop new approaches with clinical application.
Direct stem cell transplantation into the cochlea, seems to be insufficient to regenerate a substantial number of HCs or reconstruct the sensory epithelium. One possible limitation could be the restricted control of the cell progenitors differentiation. Moreover, some important aspects of the development, maturation and function of HCs are unknown. For example, the Atoh1 gene appears to be sufficient to induce HC differentiation [68]. However, the cascade of signals that distinguish the development of vestibular or cochlear HCs phenotypes have not been clarified. The identification of these factors and molecular mediators, may allow improvements in the differentiation process of iPSCs and ES toward otic lineages, in order to obtain specific cell types and have greater control over the proliferation and differentiation of progenitors transplanted into the cochlea. Nevertheless, those novel cells have to be properly organized, regarding their ultrastructure and polarity, and be able to restore the organ of Corti cytoarchitecture. All these aspects have to be further investigated.
We believe that iPSCs will have an important role for the development of stem cell-based treatments for hearing diseases. For example, patients with gradual or sudden hearing loss may benefit from iPSCs-derived HCs, along with a treatment or genetic reprogramming modulating NT-3 and/or RGMa expression to promote the formation of new synapses. However, we have only found two ongoing clinical trials regarding stem cells and hearing loss. Both trials (NCT02038972 and NCT01343394; ClinicalTrials.gov; accessed 5 September 2016) are designed to evaluate the safety of employing autologous umbilical cord blood stem cells in children with early hearing loss, following their auditory response.
Some studies have shown the utility of zebrafish as a model for screening new drugs for hearing therapy [69]. However, no drug discovered or tested in this model has yet been used in human clinical trials. Another promising model for these screenings is the iPSCs. These cells can generate different phenotypes of HCs, on which new drugs can be tested. Nevertheless, the efficiency for the otic precursors differentiation is still insufficient.
For their part, neurotrophin signaling methods inducing ribbon synapsis formation should be further studied. The neurogenesis of functional ribbon systems requires from the neurofilaments to reinnervate HCs and then differentiate to form the postsynaptic complex, both structurally and biochemically. Therefore, extensive studies are still required to reveal how neurotrophin signaling induces such specialization. This knowledge would define new targets for regenerative therapy in cochlear synapses.
Conclusions
In our opinion, hearing regeneration should be considered from a multidisciplinary point of view, not only focused on stem cells, but also considering molecular mediators as a strategy to improve the outcome. Some combined therapies have been shown to be a better approach to treat some diseases than singular therapies, for instance, stem cell delivery with gene therapy to treat critical limb ischemia [70].
As was extensively discussed in this review, the transplantation of stem cell-derived otic progenitors or adult stem cells (as NSCs), results in a significant improvement in hearing, which is especially noticeable in neuronal regeneration [35]. However, the cells have to properly migrate to the damaged area and promote the establishment of functional synaptic connections between HCs and SGNs, which could be improved with molecular mediators [8, 10, 49, 64] or genetic engineering [9]. Moreover, the reconstruction of the cytoarchitecture of the organ of Corti seems an insurmountable challenge, because of its complex structure, compartmentation, and differences in fluid composition between chambers [2, 3]. To achieve a theoretical full recovery, specific HC subtypes should be obtained and integrated in their correct and functional position [20]. Nevertheless, auditory regeneration should not be considered as a therapy with only two outcomes, full success or full failure, as the current experimental therapies have produced improvements in hearing performance (partial recoveries), without establishing an organized integration of exogenous cells, for example, with the formation of SGNs from stem cells [35].
In our opinion, stem cells can be of great interest to improve the outcome of current hearing devices. Co-therapy with otic neural precursors and cochlear implants could provide good results [45]. With this principle, stem cell therapy could increase the number of functional SGNs, as seen in the paper by Masahiro Matsumoto et al. [50], which at last may be susceptible to stimulation by the implant electrodes. For patients with SGN degeneration, the auditory brainstem implant is the only therapeutic option, but this device provides a much more limited hearing capacity, compared with cochlear implants [71]. This fact could increase the interest in the combination of cell therapy and cochlear implants in the near future.
In conclusion, there are a lot of issues in the hearing regeneration field to be solved, in order to develop new therapies that could provide the inner ear with new otic stem cells. Those stem cells delivery may be a good way to provide cells with self-renewal capacity, as they are absent in adult cochlea. They may be also a good reinforcement for the cochlear implant. However, stem cell-based clinical trials directed at hearing regeneration have to be launched to validate such a hypothesis.